In the bosonic approach the matter excitations are modelled as coupled harmonic oscillators. While this approach is very efficient to provide the energies of the collective excitations and the light-matter coupled states, it loses track of the dynamics of electronic populations that are considered as constant parameters. Besides, the energies of the collective states are renormalized by interactions and thus significantly detuned from bare electronic levels responsible for the current flow.
In the article Pisani et al. recently published in Nature Communications, the QUAD team of the LPENS address this problem by experimental and theoretical investigations of semiconductor quantum detectors where a single-particle electronic extractor level is brought into resonance with collective light-matter coupled states. They develop a quantum theory that is free from the bosonisation approach and explicitly takes into account the fermionic nature of the carriers, as well as the population dynamics, through a set of non-linear Bloch-type equations. Their theory quantitatively explains both the magnitude and the spectral features of the photocurrent (Figure), and enables understanding of how collective electronic states produce single-particle currents in quantum devices. These findings open a new way of conceiving optoelectronic devices based on the coherent interaction between electrons and photons allowing, for example, the optimization of quantum cascade detectors operating in the regime of strongly non-perturbative coupling with light.
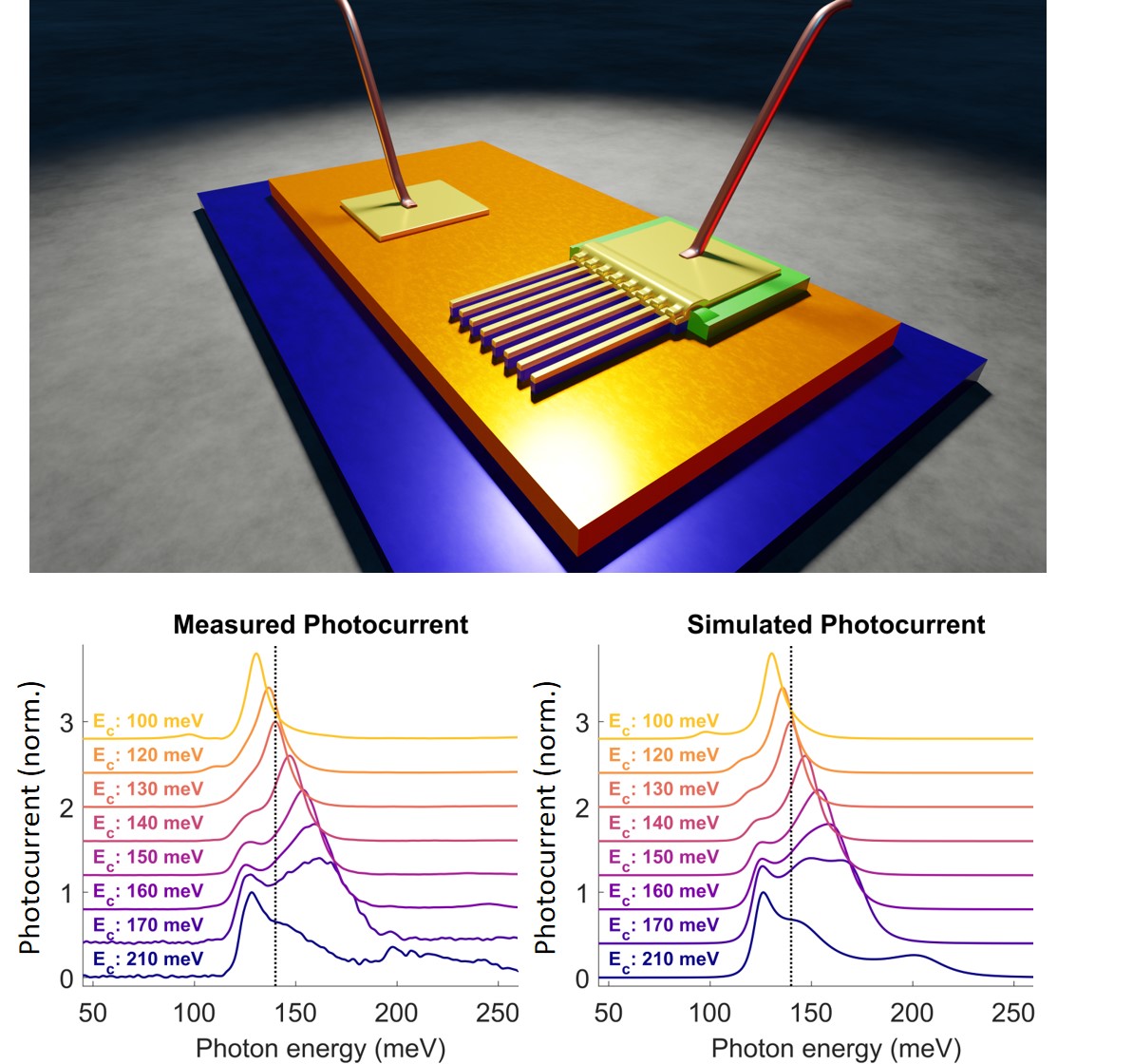
Upper panel : schematics of the quantum photodetector used in this study. Lower panel: experimental photocurrent spectra (left) and spectra reproduced by our quantum model (right). Ec designates the energy of the microcavity mode interacting with the absorbing region of the detector.
More:
https://www.nature.com/articles/s41467-023-39594-z
Affiliation author:
Laboratoire de physique de L’École normale supérieure (LPENS, ENS Paris/CNRS/Sorbonne Université/Université de Paris)
Corresponding author : Yanko Todorov
Communication contact: Communication team